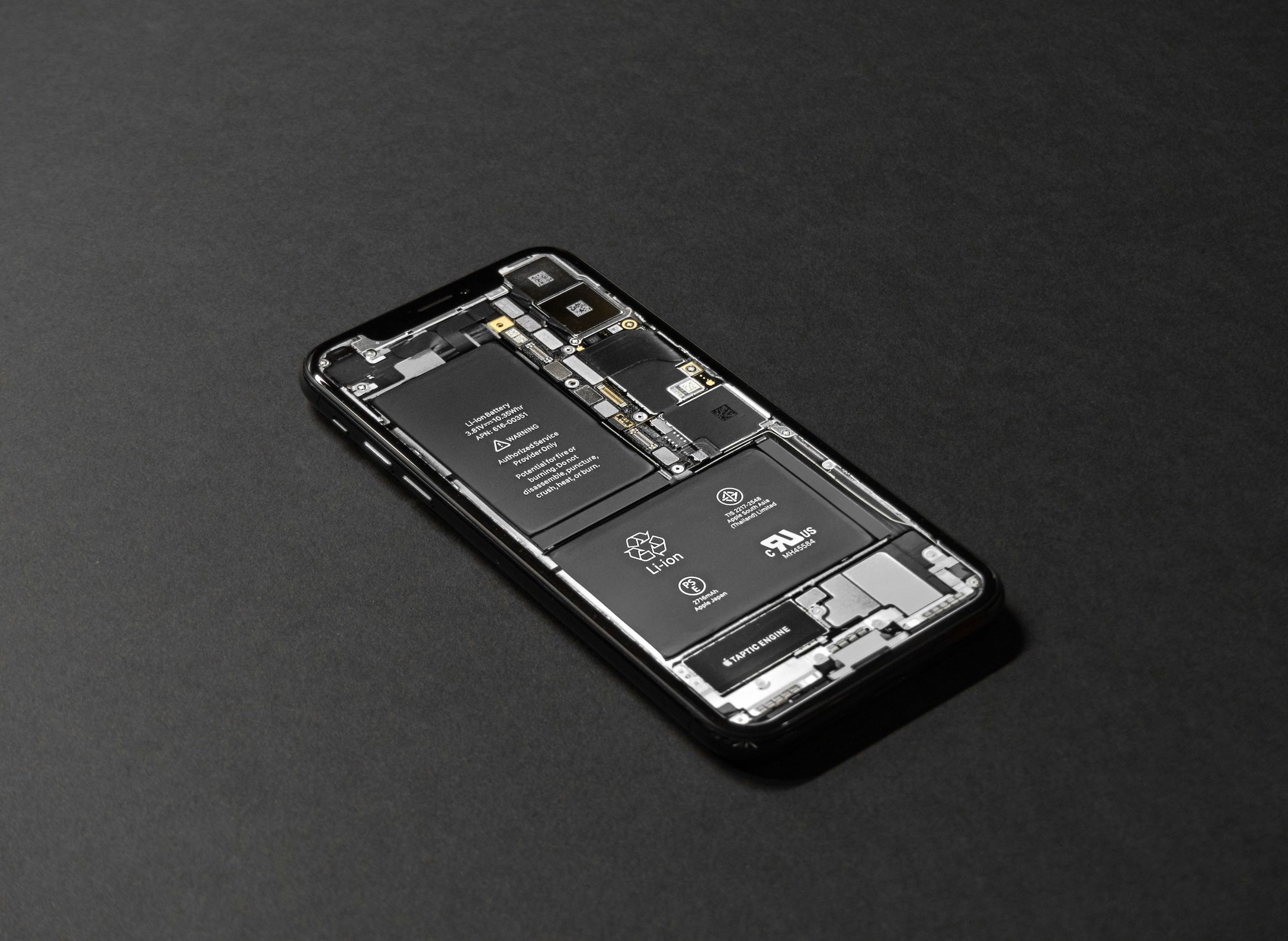
Lithium-ion batteries today are nearly ubiquitous, powering everything from cell phones to laptops. Small wonder, then, that scientists are continually trying to develop safer and more energy-efficient battery technology.
A research collaborative including UC Santa Barbara materials and mechanical engineer, battery expert Jeff Sakamoto, recently revealed key insights into solid electrolytes being tested for use in all-solid-state batteries. The paper, co-authored by researchers in the U.S. Department of Energy’s (DOE) Argonne National Laboratory and their collaborators, is published in the journal ACS Materials Letters.
Electrolytes are like membranes that allow only lithium ions (no electrons) to flow between the positive and negative electrodes of a battery. Separated from their lithium atoms, the electrons flow in an external circuit in one direction to charge the battery — and in the opposite direction to discharge it and power electrical devices. Solid-state batteries, which by definition incorporate solid, rather than liquid electrolytes, are emerging as a critical technology for developing lithium-ion batteries that are lightweight, energy-dense, longer-lasting and, critically, safer. The enhanced safety derives from the fact that solid electrolytes are neither volatile nor flammable, unlike the liquid electrolytes used in conventional lithium-ion batteries.
Solid electrolytes can be less reactive with lithium metal, making them more compatible with lithium-metal electrodes than with liquid electrolytes. Because all atoms in lithium metal can participate in the charge and discharge of a battery — enabling it to store more energy — lithium metal has a higher energy density than graphite, a conventional electrode material. Solid electrolytes made of lithium lanthanum zirconium garnet (LLZO) are a leading candidate for such a battery. This material stands out because of its stiffness, durability and conductivity; that is, the ease with which it moves lithium ions between electrodes during charge and discharge.
To make LLZO even better, researchers have been experimenting with doping — adding small amounts of such elements as aluminum or gallium — to improve its ability to conduct lithium ions.
Doping with aluminum and gallium helps LLZO, which Sakamoto produced for the study, to retain the most symmetrical structure while also creating vacant spaces. And that in turn facilitates lithium-ion motion, improving conductivity. Doping can, however, make the LLZO more reactive with lithium metal, shortening the cycle life of the battery. Optimally balancing such processes by varying the material composition of the electrolyte or other elements of a battery to gain an advantage without incurring a disadvantage lies at the very heart of battery research.
In the study, researchers examined what happens when LLZO, containing aluminum or gallium dopants, contacts metallic lithium. Using computational and experimental techniques, they found that gallium tends to move more easily out of the electrolyte and has a stronger tendency to react with the lithium to form an alloy, causing the amount of gallium to decrease. That loss of gallium can cause the lithium garnet to change its structure and decrease ionic conductivity. Conversely, LLZO doped with aluminum remains intact.
With a much higher ionic conductivity than aluminum-doped LLZO, the gallium-doped version is attractive. But the reactivity of the dopants with lithium led researchers to determine that using gallium requires an interfacial layer to protect and preserve its conductivity while preventing the associated reactivity. Understanding why the LLZO behaves differently depending on which dopant is added will help scientists design better materials for stable and reliable solid-state batteries.
“If dopants are unstable, having improved conductivity is not enough,” explained Sanja Tepavcevic, an Argonne chemist and lead experimentalist on the study, noting that separating reactivity from conductivity, or developing one material that has both high conductivity and stability, is “basically what we are trying to show with this work.”
By combining computational and experimental techniques, the researchers were able to measure key properties of the doped materials, while gaining atomic-level insights into what’s happening at the interface between the lithium metal and the solid electrolyte. Using a powerful computer-based method known as density functional theory to study how atoms and electrons behave in materials, they were able to predict the stability of various dopants and how they would react with other substances.
Few experimental techniques allow scientists to look at the solid electrolyte-electrode interface, especially while an electrochemical reaction is occurring during battery operation. That’s because these interfaces are “buried” and not visible with most experimental techniques. The researchers used X-ray photoelectron spectroscopy to study changes in the surface chemistry of LLZO and employed electrochemical impedance spectroscopy to analyze the movement of lithium ions in electrolytes and at the electrolyte-electrode interface. They also used neutron diffraction to determine how atoms are arranged in a material. The researchers were able to confirm that gallium became less stable and more reactive once it interacted with lithium, whereas aluminum remained stable.